One spring day 66.043 million years ago, a meteorite smashed into limestone at the ocean’s edge near the present-day Yucatán Peninsula.
The rock, at least six miles in width, rushed toward the planet at 46,000 miles per hour, too fast to have been seen by the dinosaurs it annihilated. Shattering rocks to a depth of 18 miles, it unleashed a tsunami and set wildfires for hundreds of miles around. A thousand miles from the site of impact, a cloud of gas heated to 311°F swept across the Yucatán shoreline.
Vast amounts of rock blasted into the stratosphere and rained back down as molten droplets, called tektites, heating the planet’s atmosphere and roasting animals that could not find shelter in water or deep burrows. Sulfur unleashed from shattered limestone combined with oxygen to form thick clouds that dimmed the sun’s rays and set off storms of acid rain around the globe. Ecosystems that survived the initial impact collapsed.
The chain reaction set off by the asteroid strike eventually snuffed out 99.9999% of life on the planet. The ash and bits of meteorite left behind would settle into a thin, distinctive gray line in the earth, marking a mass grave and the dividing line between periods of life on the planet.
How do we know such precise details about the worst day in Earth’s history so many millions of years ago?
Partly it comes from scientists working together across disciplines. What began as a paleontological puzzle attracted the expertise of ecologists, physicists, computer modelers, and atmospheric scientists. Some of the most surprising discoveries came from nuclear chemistry, a discipline whose origins can be traced to Henri Becquerel’s 1896 discovery of radioactivity. By decoding the chemical remnants of ancient events, scientists have probed the planet’s primordial history in often mind-boggling detail.
Such achievements, however, were neither planned nor predictable in 1896. They were reached only after a series of twists and turns navigated by generations of scientists, some poring over the latest electronic innovations, others interrogating peculiar rock samples from around the world.
How Old Is Earth?
“It is perhaps a little indelicate to ask of our Mother Earth her age,” wrote Arthur Holmes in 1913. But Holmes, eventually recognized as the father of the geologic timescale, was nearly alone in his bashfulness. As geologist Brent Dalrymple makes clear in The Age of the Earth, people had had the effrontery to ask Earth her age for thousands of years.
The ancient Hindus, for example, calculated the world to be about two billion years old. The Chaldean rulers of the Neo-Babylonian Empire in the period 612 to 538 BCE held that Earth had emerged from chaos more than two million years earlier.
Others pegged Earth as far younger. Most likely during the 2nd millennium BCE, Persian religious reformer Zoroaster put the estimate at 12,000 years old; Hebrew and Christian calculations produced ages that were younger still. The probable founder of the Christian chronological tradition, Syrian saint and Christian apologist Theophilus of Antioch (ca. 115–180), estimated Earth had been created 5,732 years earlier (5529 BCE), based on a study of Scripture. Subsequent Christian estimates, drawing on various biblical texts, all attributed a young age to Earth, around 6,000 years. Since time in the Bible is measured by day or generation, Christian chronologists typically estimated the time elapsed between milestones, such as the Flood or the birth of Abraham, by adding up generations and the reigns of various rulers.
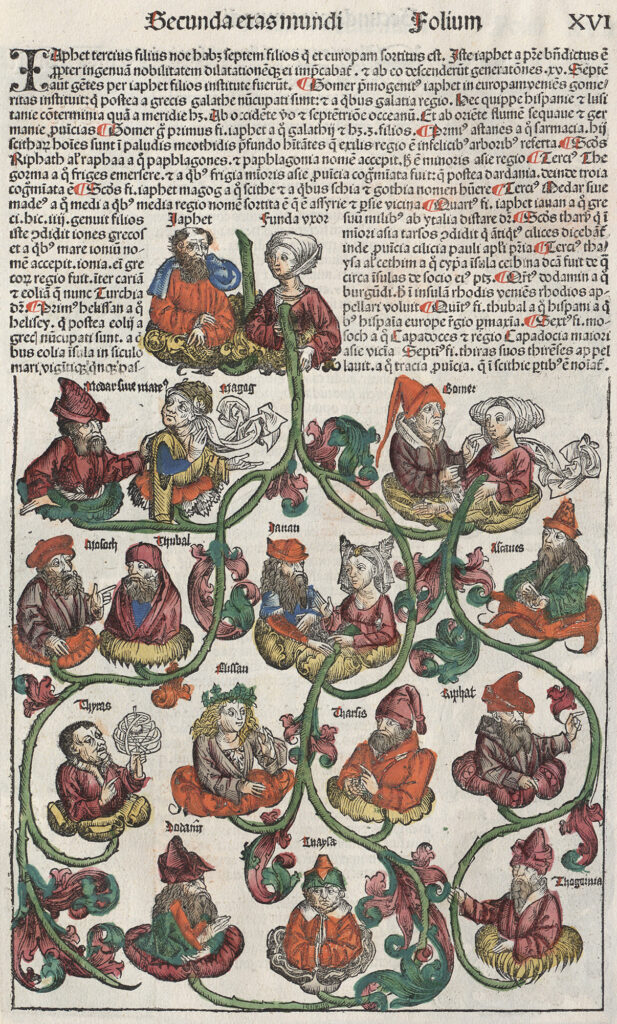
In the early modern period, natural philosophers began incorporating non-biblical data from astronomy—the most prestigious science at the time—into their estimates. Thus, Johannes Kepler (1571–1630), the German discoverer of the planets’ elliptical orbits, used orbital data to calculate that Earth was created in 3993 BCE. Prelate and biblical scholar James Ussher (1581–1656), combined biblical sources, historical accounts, and data on astronomical cycles to estimate a creation date of 4004 BCE.
In the West, the first estimate based solely on data from nature was made by French diplomat and amateur naturalist Benoît de Maillet (1656–1738). De Maillet’s calculation assumed sea levels had been declining steadily since Earth’s beginning. This assumption was based on the discovery of seashells in inland mountains and the ideas of de Maillet’s philosophical guru, René Descartes, who held that the planets were swirling in a vortex and could spin off water in the melee. Estimating the rate of ocean decline at 3 inches per century, de Maillet concluded Earth was at least two billion years old. This age represented a radical departure from the youthful Earth determined from biblical sources and launched the modern practice of scientific age determination.
The use of a continuous natural process—declining sea levels, in de Maillet’s case—as a clock to measure Earth’s age became the standard approach in the 18th and 19th centuries. Other clocks included salt accumulation in the oceans; the slowing of Earth’s rotation through tidal dissipation of energy; sediment accumulation, based on measuring the thicknesses of rock strata; and the alleged cooling of Earth since an early molten state, an idea proposed by physicist Lord Kelvin. Though these scientific estimates varied greatly in approach, they all attributed much older ages to Earth than had the Scripture-based chronologies.
The planet’s age was of particular importance to early geologists as their field took form in the 18th and 19th centuries. Variations in rock strata and fossils appeared to mark different periods and transitions in the planet’s geological activity, climate, and the life forms it supported. But without a definitive age, how could geologists know they had accounted for all such divisions since Earth formed? Conversely, when they attempted to calculate the durations of these divisions, what could they use to check their math?
The age of Earth would serve as a useful constraint to address both questions: the sum of geologic eras could not be greater than Earth’s total age, and conversely, if Earth’s age were greater than the sum of all known geologic eras, it followed that some eras had not yet been discovered. Thus, the millennia-spanning, largely religious quest to date the planet gained a scientific impetus.
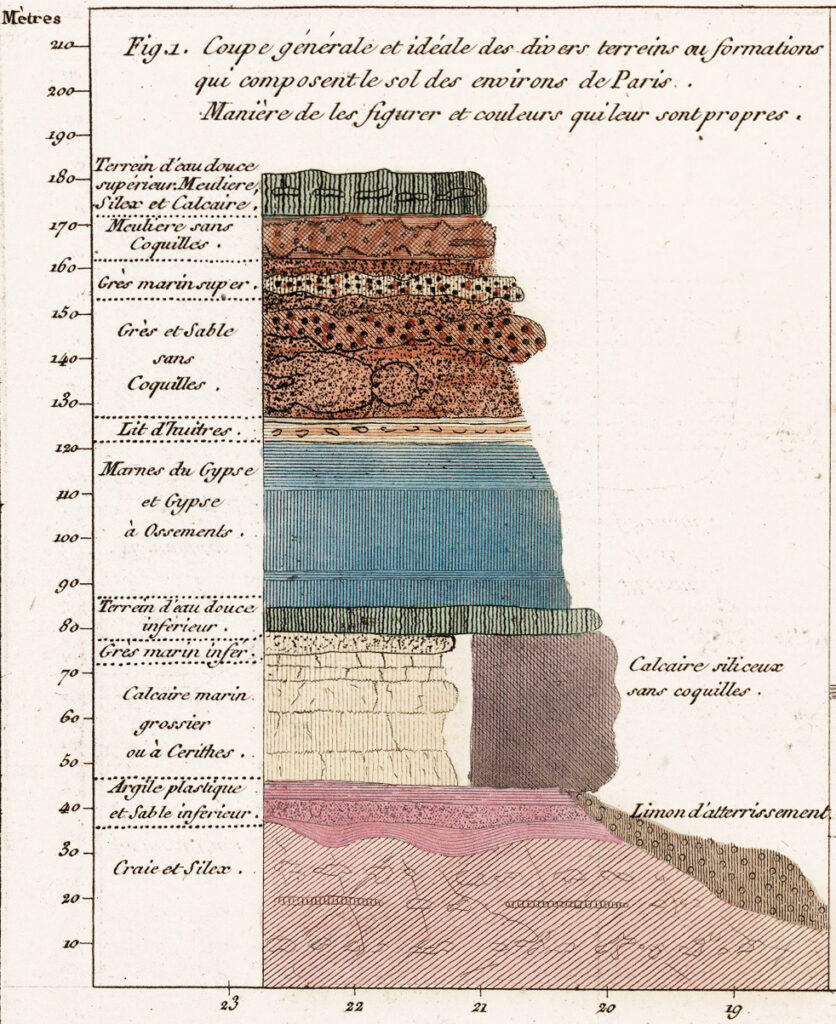
Geologists’ preferred method for measuring geological time, including the age of Earth, involved the study of sedimentary rock. Sediment accumulation means geologic activity—the ticking of the clock. Over time, sediments are deposited on the floors of aquatic bodies. This process is offset by erosion. The overall rate of accumulation represents the balance between deposition and erosion.
With a reliable rate of accumulation, it would be a relatively simple task for geologists to measure deposits and calculate the total time since sediments had started forming in the oceans and seas and thus establish a lower limit on Earth’s age. But no one could agree on what that rate should be. Estimates varied wildly depending on which rock formations and accumulation rates were used as inputs. The only thing geologists could agree on was that Earth was very, very old, on the order of 100 million years, if not much older.
The study of sedimentary rock appealed to geologists, at least in part, because they could use data and concepts from their own field rather than borrow them from physics or chemistry, as ocean-salt accumulation and other methods had. But the existence of these alternatives, as well as the obvious inconclusiveness of the geologists’ preferred method, stoked interdisciplinary tensions.
Ever since James Hutton published his 1788 manifesto for uniformitarianism—the theory that Earth had been shaped not by the cataclysmic events noted in Scripture but by the gradual effects of processes like those we see today—geologists had granted themselves large timespans to account for the thick accumulations of sedimentary rocks and for the numerous evolutionary changes evident in the fossil record. As Hutton famously put it in the modestly titled Theory of the Earth, “the result . . . of our present enquiry is that we find no vestige of a beginning, no prospect of an end.”
The trouble started when, in the late 19th century, the world’s most famous physicist, Lord Kelvin boldly proclaimed that his calculations proved Earth could not be more than 24 million years old. This claim flew in the face of geological evidence and evolutionary theory. As a result, geologists scorned the theory and resisted the intrusion of physical methods onto their turf for decades.
Kelvin’s calculations were based on his research in thermodynamics. He argued that all the energy in the solar system, including that contained in the sun and Earth, would necessarily dissipate over time. The celestial bodies were like glowing coals slowly cooling in the void of space. It followed that Earth had been hottest at the time of formation, and so its age could be estimated based on hypotheses, which Kelvin put forth, about the rate of cooling.