This year marks the 100th anniversary of the death of one of the most famous scientists of all time, the Russian chemist Dmitri Ivanovich Mendeleev (1834–1907). The periodic table that he introduced in 1869 was a monumental achievement—a wonderful mnemonic and a tool that serves to organize the whole of chemistry. No longer were students of chemistry obliged to memorize the properties of all the known elements; hereafter they could learn the properties of at least one element from each column and could, in principle, make sound predictions about the other group members.
Arguably, however, Mendeleev’s greatest achievement was not the periodic table so much as the recognition of the periodic system on which it was based. Of the nearly 1,000 variations that have been published since, all are attempts to represent the fundamental rule that after certain but varying intervals the chemical elements show an approximate repetition in their properties.
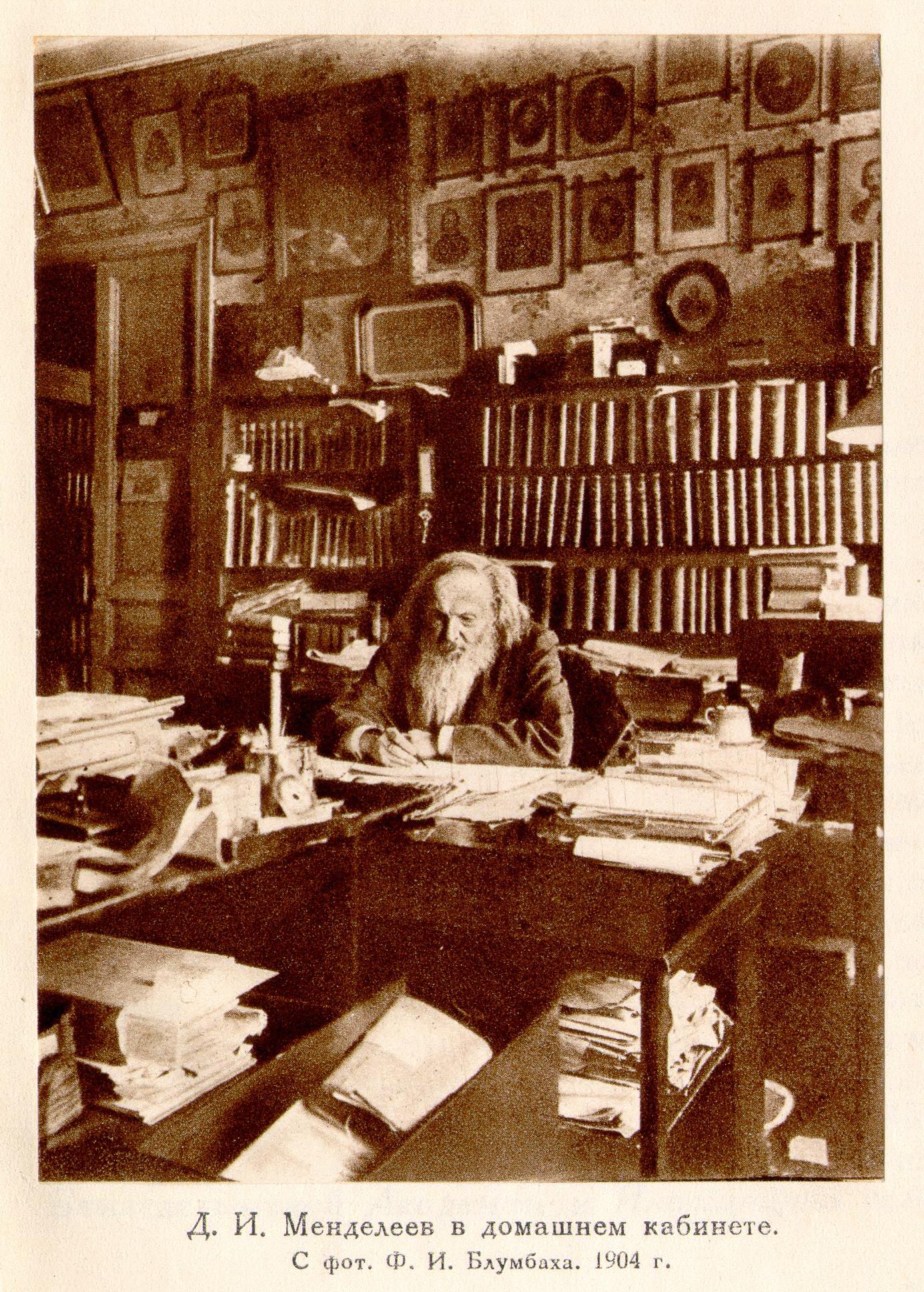
Mendeleev was hardly the first to arrive at a periodic system. The observation that certain types of elements prefer to combine with certain other types prompted early chemists to classify the elements in tables of chemical affinities. In 1817 the German chemist Johann Wolfgang Döbereiner noticed the existence of groupings of elements in threes, subsequently called triads. The elements in these groupings displayed an important numerical relationship to each other: the equivalent weight (an early substitute for atomic weight) of the middle element had the approximate mean of the values of the two flanking elements. Although Döbereiner worked with the rather crude approximations of atomic weight available at the time, he successfully identified four such groups: strontium, calcium, and barium; bromine, iodine, and chlorine; sodium, lithium, and potassium; and selenium, sulfur, and tellurium. His triads—which would eventually appear on the periodic table in vertical columns—represented the first step in fitting the elements into a system that would account for their chemical properties and reveal their physical relationships.
By the 1860s a number of scientists had moved beyond the triad concept to produce some very respectable periodic systems. The French geologist Alexandre-Émile Béguyer de Chancourtois achieved the first true periodic system in 1862 by arranging the elements by atomic weight in a spiral line wrapped around a metal cylinder. Periodic relationships could be seen by moving vertically down the screw. In 1863 and 1864 two British chemists, John Newlands and William Olding (coincidentally, both born in the same London borough of Southwark), independently published periodic tables that used atomic weight to arrange the elements into groups with analogous properties. A more eccentric spiral periodic system was created by the Danish-born polymath Gustavus Hinrichs in 1864. Hinrichs was intrigued that atomic spectral frequencies, like planetary distances, show whole number ratios, and he concluded that atomic spectra must therefore be an indication of atomic size.
The closest precursor to Mendeleev’s table in both chronological and philosophical terms was developed by Julius Lothar Meyer, a German chemist, in 1864.
Although Meyer stressed physical rather than chemical properties, his table bears remarkable similarity to the one that Mendeleev would develop five years later. For a number of reasons, Meyer’s prominence in the history books never matched Mendeleev’s. There was an untimely delay in the publication of his most elaborate periodic table, and, perhaps more importantly, Meyer—unlike Mendeleev—hesitated to make predictions about unknown elements.
Notwithstanding these earlier scientists’ contributions to the idea of periodicity, Mendeleev remains the undisputed champion of the periodic system in the literal sense of its defense, propagation, and elaboration. Mendeleev’s version of the periodic table left the biggest impact on the scientific community, both at the time it was produced and thereafter. In the popular imagination the periodic system invariably and justifiably connects to his name, to the same extent that the theory of evolution connects to Darwin’s name and the theory of relativity to Einstein’s. But what really set Mendeleev’s contribution apart?
From Simple Substances to Abstract Elements
By organizing the elements as he did Mendeleev took a stand on the centuries-old question of the philosophical status of the elements. Unlike some of his contemporaries Mendeleev rejected the suggestion that the periodic system implied the existence of any form of primary matter of which all the elements were composed. He maintained that all elements were strictly individual, indestructible, and irreducible, yet he acknowledged the seeming challenge posed by chemical reactions. Consider the familiar example of sodium chloride: common white table salt does not seem to include either poisonous grey metallic sodium or poisonous green gaseous chlorine.
Aristotle had maintained that all matter was composed of some combination of four abstract elements: earth, fire, water, and air. Although the four elements were themselves unobservable, their relative proportions within a specific substance governed its properties. Antoine-Laurent Lavoisier and his contemporaries challenged this view in the 18th century with new concepts of simple substances. They created a list of 37 simple substances that could be isolated from the decomposition of compounds and could not be further decomposed by any known means.More than any of the other discoverers of the periodic system, Mendeleev was concerned with the philosophical status of the elements. At the beginning of the first volume of his landmark Principles of Chemistry (first English translation, New York, 1891; Osnovy Khimii, first edition, Saint Petersburg, 1869), he wrote, “It is useful in this sense to make a clear distinction between the conception of an element as a separate homogenous substance and as a material but invisible part of a compound” (p. 23). For Mendeleev an element was an entity that was essentially unobservable but formed the inner essence of simple bodies. Whereas a particular element was to be regarded as unchanging, its corresponding simple-body aspect could take many forms such as charcoal, diamond, and graphite in the case of carbon. His periodic table classified abstract elements, not simple substances.
Mendeleev’s genius lay in recognizing that just as it was the element in the abstract sense that survived intact in the course of compound formation, so atomic weight was the only quantity that survived in measurable amounts. He therefore took the step of associating these two features: an element—a —basic substance—was to be characterized by its atomic weight. In a sense an abstract element had acquired a single measurable attribute that would remain unchanged in all its chemical combinations. Here, then, was a profound justification for using atomic weight as the basis for the classification of the elements, unlike any of the precursors to the periodic system.
Isotopes
Toward the end of Mendeleev’s life a growing body of evidence began to challenge his conception of the nature of the elements. Several revolutionary discoveries in physics showed that atoms were, in fact, reducible, and that there was a sense in which all elements are composed of the same primary matter: protons, neutrons, and electrons. Most alarmingly, there was even evidence to suggest that certain elements could be transformed into others through radioactivity.
In 1879 J. J. Thomson identified electrons as the particles constituting cathode rays. By repeating his experiments with cathode rays produced by different elements, he concluded that the same particle was produced in every case, and that this particle was therefore a fundamental constituent of all matter. Shortly thereafter Henri Becquerel and the Curies began to explore the phenomenon of radioactivity. One of the most talented researchers attracted to the study of radioactivity was Ernest Rutherford, who suggested in 1902 that radioactive reactions had the power to transform certain elements into entirely different elements. While fully aware of the possible criticism that such a notion might bring, Rutherford and his colleague Frederick Soddy went so far as to describe this new phenomenon as chemical transmutation, thus evoking the age-old dream of the alchemists.
As if the threat of transmutation were not enough, the discovery of isotopy nearly unraveled the entire periodic system. Scientists’ failure to separate radioisotopes by chemical means threatened both the traditional notion of the elements and the utility of atomic weight as an elemental characteristic. Reflecting on this sad situation in the Annual Report to the London Chemical Society in 1911, Soddy wrote, “Chemical homogeneity is no longer a guarantee that any supposed element is not a mixture of several different atomic weights, or that any atomic weight is not merely a mean number. The constancy of atomic weight, whatever the source of the material, is not a complete proof of homogeneity.”
This situation was clarified somewhat when Soddy and Kasimir Fajans, a Polish-born chemist, independently suggested what became known as the group displacement laws in 1913. Each found that the emission of an alpha particle from an element produces an element located two places to the left on the periodic table, while the emission of a beta particle resulted in a movement one position to the right. For example if an atom of uranium-235 undergoes alpha decay, it forms an atom of thorium-231; meanwhile, an atom of actinium-230 can undergo a beta decay to form an atom of thorium-230. Today we would recognize both products as atoms of the same elements with different atomic weights, but at the time the matter of elemental identity had not yet been settled.
By the 1920s the periodic system was in crisis. Many new isotopes had been discovered over a short period of time so that the number of “atoms,” or fundamental units, suddenly seemed to have multiplied. At this point some chemists, including Fajans, called for abandoning Mendeleev’s periodic system in favor of a more complicated table of isotopes. A way out appeared in the form of atomic numbers assigned by nuclear charge. The Austrian chemist Fritz Paneth championed the atomic number—instead of atomic weight—as the primary characteristic of the elements. Paneth, along with Hungarian chemist György Hevesy, showed that the chemical properties of isotopes of the same element were, for all intents and purposes, identical. Chemists could therefore regard the isotopes of any element as being the same simple substance, even though individual atoms might appear in different isotopic forms.
Electrons
If the discovery of isotopes threatened to undermine the periodic system, the discovery of the electron explained many of the periodic properties on which the table was based. J. J. Thomson attempted to explain the periodic system by postulating rings of electrons embedded in the positive charge that comprised his plum pudding model of the atom. Thomson’s model was quickly superseded by more sophisticated and elaborate models of atomic structure. However, the origin of our current conception of electronic configurations—an explanatory paradigm in much of chemistry—can be traced to his ideas. Because of Thompson we know that the key to an atom’s properties lies in the number of outer-shell electrons, and that in turn can be deduced—with some exceptions—from an element’s position on the periodic table.
The origin of electronic configuration is frequently and inaccurately attributed to Niels Bohr, who introduced quantum theory to the study of the atom. But Bohr essentially tidied up Thomson’s pre-quantum configurations and took advantage of a more accurate knowledge of the number of electrons each of the elements actually possessed. Further developments in quantum theory, including Pauli’s exclusion principle and Schrödinger’s equation, led to a more rigorous theoretical explanation of the form of the periodic system.
Collectively, these theories established the basic principles by which electronic configurations are assigned: as one moves from left to right on the periodic table, each element contains one additional electron (Bohr’s aufbau principle); each additional electron, with certain exceptions, is added to the atom’s outermost electron shell; only two electrons can fill a single orbital; when electrons fill orbitals of equal energy, they occupy as many different orbitals as possible; and no two electrons in an atom can share the same set of quantum numbers. The rules that govern the assignment of quantum numbers are rigorously explained by quantum theory, with the outcome that the first two shells contain a maximum of two and eight electrons—at long last an explanation for the lengths of the first two periods of the table! Similar considerations for the third and fourth shells predict 18 and 32 electrons respectively, but this is not in accordance with the arrangement of the elements in the periodic table.
The problem is this: the third row of the period table contains 8, not 18, electrons. It turns out that while quantum numbers provide a satisfying deductive explanation of the total number of electrons that any shell can hold, the correspondence of these values with the number of elements that occur in any particular period is something of a coincidence. The familiar sequence in which the s, p, d, and f orbitals are filled has essentially been determined by empirical means. Indeed, Bohr’s failure to derive the order for the filling of the orbitals has been described by some as one of the outstanding problems of quantum mechanics.
The simple textbook explanation—that orbitals are filled in order of their relative energies —has its limitations, as illustrated by the cases of chromium and copper. Both copper and chromium are anomalies in that electrons are added to the 3d orbital before the 4s orbital is closed; that is, their outer shells have configurations of 4s13d5 and 4s13d10 instead of the expected 4s23d4 and 4s23d9. Scholars have offered various simple explanations for this experimental finding, the most common of which is that the stability of a half-filled or fully-filled d shell offers the most energetically stable arrangement of electrons. However, since the configurations of the elements in the second transition series follow an even more “anomalous” pattern—not necessarily involving half-filled or fully filled shells—it is clear that the explanation is specific to chromium and copper. Quantum mechanics can generally be used to explain a particular atom’s empirical electronic configuration but that configuration usually cannot be deduced from quantum mechanics alone.
It is something of a miracle that quantum mechanics explains the period table to the extent that it does; we should not let this fact seduce us into believing that it is a deductive explanation. Attempts to explain the details of the periodic table continue to challenge the ingenuity of quantum physicists and quantum chemists, and the periodic table will continue to present a test case for the adequacy of new methods developed in quantum chemistry.
A Lasting Legacy
Our story has now been brought up to date. From its humble beginnings as a set of isolated triads of elements, the periodic system has grown to embody well over 100 elements, and it has survived the discovery of isotopes and the quantum revolution in the study of matter. Rather than being swept aside, it has continued to provide a challenge to the development of ever-more accurate means of calculating the basic properties of the atoms of the chemical elements. A century’s worth of science has consolidated, rather than chipped away at the periodic system’s central role in modern chemistry.
The problem is no longer the validity of Mendeleev’s system, but the best way to represent it. Should it be the original short-form table with 8 columns, the familiar medium-long form with 18 column, or perhaps even a long-form table with 32-column, which more naturally accommodates the rare earth elements into the main body of the table? Alternatively, some favor pyramidal tables while others advocate the left-step form proposed by Charles Janet in the 1920s. Theodor Benfey and Philip Stewart have proposed continuous spiral models. Hundreds, possibly even thousands, of periodic systems have been proposed, and each has its ardent supporters.
Is there one best periodic table? Many chemists argue that the form of the table is of little importance, and that one’s choice depends on what particular aspect of periodicity one wants to depict. But surely this is not the case if, for example, rival versions put helium and hydrogen in radically different places. Such debates will continue for a long time. However, the debate would not exist with out Dmitri Ivanovich Mendeleev, and for the very legacy of periodicity, we are indebted to him.